Paper:
Analysis of Inward Vascular Remodeling Focusing on Endothelial–Perivascular Crosstalk in a Microfluidic Device
Ryosuke Murai*, Masafumi Watanabe*
, and Ryo Sudo*,**

*School of Integrated Design Engineering, Graduate School of Science and Technology, Keio University
3-14-1 Hiyoshi, Kohoku-ku, Yokohama, Kanagawa 223-8522, Japan
**Department of System Design Engineering, Facluty of Science and Technology, Keio University
3-14-1 Hiyoshi, Kohoku-ku, Yokohama, Kanagawa 223-8522, Japan
Vascular remodeling is a crucial process for the effective delivery of oxygen and nutrients to the entire body during vascular formation. However, detailed mechanisms underlying vascular remodeling are not yet fully understood owing to the absence of an appropriate experimental model. To address this, in this study, we utilized a microfluidic vascular model with perivascular cells to investigate the mechanism of vascular remodeling by culturing human umbilical vein endothelial cells (HUVECs) and mesenchymal stem cells (MSCs) in a microfluidic device. We compared two different cell culture conditions: culturing HUVECs and MSCs (1) separately in different channels and (2) in the same channel. In both conditions, microvascular networks covered with perivascular cells were formed. Interestingly, a significant inward vascular remodeling occurred over time when HUVECs and MSCs were cultured in different channels. This remodeling was mediated by direct endothelial–perivascular crosstalk through α6 integrin. Furthermore, computational fluid analysis revealed that hypothetical shear stress on the luminal surface of microvessels was attenuated during inward vascular remodeling, suggesting that the remodeling might be an adaptive change. Our findings and the microfluidic model will be useful not only for further elucidation of mechanisms underlying physiological and pathological vascular remodeling but also for constructing functional vascularized tissues and organs by controlling vascular remodeling.
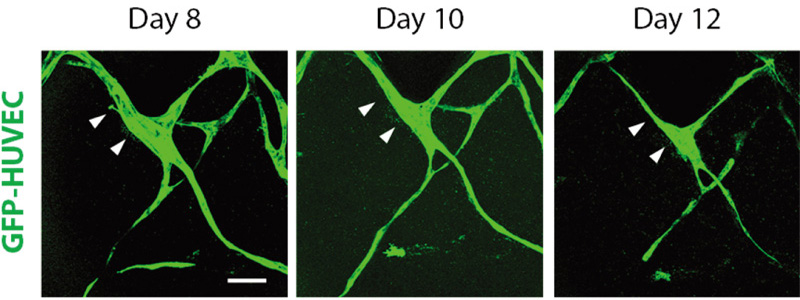
Live cell imaging of inward vascular remodeling
- [1] A. R. Pries and T. W. Secomb, “Making microvascular networks work: Angiogenesis, remodeling, and pruning,” Physiology, Vol.29, No.6, pp. 446-455, 2014. https://doi.org/10.1152/physiol.00012.2014
- [2] R. Santamaría, M. González-Álvarez, R. Delgado, S. Esteban, and A. G. Arroyo, “Remodeling of the microvasculature: May the blood flow be with you,” Front. Physiol., Vol.11, Article No.586852, 2020. https://doi.org/10.3389/fphys.2020.586852
- [3] C. M. Peppiatt, C. Howarth, P. Mobbs, and D. Attwell, “Bidirectional control of CNS capillary diameter by pericytes,” Nature, Vol.443, No.7112, pp. 700-704, 2006. https://doi.org/10.1038/nature05193
- [4] A. L. Gonzales et al., “Contractile pericytes determine the direction of blood flow at capillary junctions,” Proc. Natl. Acad. Sci., Vol.117, No.43, pp. 27022-27033, 2020. https://doi.org/10.1073/pnas.1922755117
- [5] M. R. Ward et al., “Low blood flow after angioplasty augments mechanisms of restenosis: Inward vessel remodeling, cell migration, and activity of genes regulating migration,” Arterioscler. Thromb. Vasc. Biol., Vol.21, No.2, pp. 208-213, 2001. https://doi.org/10.1161/01.ATV.21.2.208
- [6] M. Sigovan et al., “Vascular remodeling in autogenous arterio-venous fistulas by MRI and CFD,” Ann. Biomed. Eng., Vol.41, No.4, pp. 657-668, 2013. https://doi.org/10.1007/s10439-012-0703-4
- [7] Y. Shin et al., “Microfluidic assay for simultaneous culture of multiple cell types on surfaces or within hydrogels,” Nat. Protoc., Vol.7, No.7, pp. 1247-1259, 2012. https://doi.org/10.1038/nprot.2012.051
- [8] W. J. Polacheck, M. L. Kutys, J. B. Tefft, and C. S. Chen, “Microfabricated blood vessels for modeling the vascular transport barrier,” Nat. Protoc., Vol.14, No.5, pp. 1425-1454, 2019. https://doi.org/10.1038/s41596-019-0144-8
- [9] R. K. Jain, P. Au, J. Tam, D. G. Duda, and D. Fukumura, “Engineering vascularized tissue,” Nat. Biotechnol., Vol.23, No.7, pp. 821-823, 2005. https://doi.org/10.1038/nbt0705-821
- [10] G. Simitian, M. Virumbrales-Muñoz, C. Sánchez-de-Diego, D. J. Beebe, and D. Kosoff, “Microfluidics in vascular biology research: A critical review for engineers, biologists, and clinicians,” Lab Chip, Vol.22, No.19, pp. 3618-3636, 2022. https://doi.org/10.1039/D2LC00352J
- [11] J. W. Song and L. L. Munn, “Fluid forces control endothelial sprouting,” Proc. Natl. Acad. Sci., Vol.108, No.37, pp. 15342-15347, 2011. https://doi.org/10.1073/pnas.1105316108
- [12] J. W. Song, D. Bazou, and L. L. Munn, “Anastomosis of endothelial sprouts forms new vessels in a tissue analogue of angiogenesis,” Integr. Biol., Vol.4, No.8, pp. 857-862, 2012. https://doi.org/10.1039/c2ib20061a
- [13] J. H. Yeon, H. R. Ryu, M. Chung, Q. P. Hu, and N. L. Jeon, “In vitro formation and characterization of a perfusable three-dimensional tubular capillary network in microfluidic devices,” Lab Chip, Vol.12, No.16, pp. 2815-2822, 2012. https://doi.org/10.1039/C2LC40131B
- [14] Y.-H. Hsu et al., “Full range physiological mass transport control in 3D tissue cultures,” Lab Chip, Vol.13, No.1, pp. 81-89, 2013. https://doi.org/10.1039/C2LC40787F
- [15] X. Wang et al., “Engineering anastomosis between living capillary networks and endothelial cell-lined microfluidic channels,” Lab Chip, Vol.16, No.2, pp. 282-290, 2016. https://doi.org/10.1039/C5LC01050K
- [16] Y. Nashimoto et al., “Integrating perfusable vascular networks with a three-dimensional tissue in a microfluidic device,” Integr. Biol., Vol.9, No.6, pp. 506-518, 2017. https://doi.org/10.1039/c7ib00024c
- [17] Y. Abe et al., “Balance of interstitial flow magnitude and vascular endothelial growth factor concentration modulates three-dimensional microvascular network formation,” APL Bioeng., Vol.3, No.3, Article No.036102, 2019. https://doi.org/10.1063/1.5094735
- [18] K. Yamamoto et al., “The stabilization effect of mesenchymal stem cells on the formation of microvascular networks in a microfluidic device,” J. Biomech. Sci. Eng., Vol.8, No.2, pp. 114-128, 2013. https://doi.org/10.1299/jbse.8.114
- [19] K. Yamamoto et al., “Construction of continuous capillary networks stabilized by pericyte-like perivascular cells,” Tissue Eng. A, Vol.25, Nos.5-6, pp. 499-510, 2019. https://doi.org/10.1089/ten.tea.2018.0186
- [20] M. Watanabe and R. Sudo, “Establishment of an in vitro vascular anastomosis model in a microfluidic device,” J. Biomech. Sci. Eng., Vol.14, No.3, Article No.18-00521, 2019. https://doi.org/10.1299/jbse.18-00521
- [21] H. Uwamori, Y. Ono, T. Yamashita, K. Arai, and R. Sudo, “Comparison of organ-specific endothelial cells in terms of microvascular formation and endothelial barrier functions,” Microvasc. Res., Vol.122, pp. 60-70, 2019. https://doi.org/10.1016/j.mvr.2018.11.007
- [22] Y. Mabuchi et al., “LNGFR+THY-1+VCAM-1hi+ cells reveal functionally distinct subpopulations in mesenchymal stem cells,” Stem Cell Rep., Vol.1, No.2, pp. 152-165, 2013. https://doi.org/10.1016/j.stemcr.2013.06.001
- [23] D. Ribatti, B. Nico, and E. Crivellato, “The role of pericytes in angiogenesis,” Int. J. Dev. Biol., Vol.55, No.3, pp. 261-268, 2011. https://doi.org/10.1387/ijdb.103167dr
- [24] S. A. Williams et al., “Dynamic measurement of human capillary blood pressure,” Clin. Sci., Vol.74, No.5, pp. 507-512, 1988. https://doi.org/10.1042/cs0740507
- [25] P. C. Stapor, W. Wang, W. L. Murfee, and D. B. Khismatullin, “The distribution of fluid shear stresses in capillary sprouts,” Cardiovasc. Eng. Technol., Vol.2, No.2, pp. 124-136, 2011. https://doi.org/10.1007/s13239-011-0041-y
- [26] K. M. Gray and K. M. Stroka, “Vascular endothelial cell mechanosensing: New insights gained from biomimetic microfluidic models,” Semin. Cell Dev. Biol., Vol.71, pp. 106-117, 2017. https://doi.org/10.1016/j.semcdb.2017.06.002
- [27] K. Gaengel, G. Genové, A. Armulik, and C. Betsholtz, “Endothelial-mural cell signaling in vascular development and angiogenesis,” Arterioscler. Thromb. Vasc. Biol., Vol.29, No.5, pp. 630-638, 2009. https://doi.org/10.1161/ATVBAHA.107.161521
- [28] Y.-H. Wang, D.-B. Wu, B. Chen, E.-Q. Chen, and H. Tang, “Progress in mesenchymal stem cell-based therapy for acute liver failure,” Stem Cell Res. Ther., Vol.9, No.1, Article No.227, 2018. https://doi.org/10.1186/s13287-018-0972-4
- [29] N. P. Staff, D. T. Jones, and W. Singer, “Mesenchymal stromal cell therapies for neurodegenerative diseases,” Mayo Clin. Proc., Vol.94, No.5, pp. 892-905, 2019. https://doi.org/10.1016/j.mayocp.2019.01.001
- [30] D. Jovic et al., “A brief overview of global trends in MSC-based cell therapy,” Stem Cell Rev. Rep., Vol.18, No.5, pp. 1525-1545, 2022. https://doi.org/10.1007/s12015-022-10369-1
- [31] H. Kamihata et al., “Implantation of bone marrow mononuclear cells into ischemic myocardium enhances collateral perfusion and regional function via side supply of angioblasts, angiogenic ligands, and cytokines,” Circulation, Vol.104, No.9, pp. 1046-1052, 2001. https://doi.org/10.1161/hc3501.093817
- [32] N. F. Renna, N. de las Heras, and R. M. Miatello, “Pathophysiology of vascular remodeling in hypertension,” Int. J. Hypertens., Vol.2013, Article No.808353, 2013. https://doi.org/10.1155/2013/808353
- [33] N. B. Hamilton, D. Attwell, and C. N. Hall, “Pericyte-mediated regulation of capillary diameter: A component of neurovascular coupling in health and disease,” Front. Neuroenergetics, Vol.2, Article No.5, 2010. https://doi.org/10.3389/fnene.2010.00005
- [34] Q. Shen et al., “Adult SVZ stem cells lie in a vascular niche: A quantitative analysis of niche cell-cell interactions,” Cell Stem Cell, Vol.3, No.3, pp. 289-300, 2008. https://doi.org/10.1016/j.stem.2008.07.026
- [35] B. Carrion, Y. P. Kong, D. Kaigler, and A. J. Putnam, “Bone marrow-derived mesenchymal stem cells enhance angiogenesis via their α6β1 integrin receptor,” Exp. Cell Res., Vol.319, No.19, pp. 2964-2976, 2013. https://doi.org/10.1016/j.yexcr.2013.09.007
- [36] L. E. Reynolds et al., “Dual role of pericyte α6β1-integrin in tumour blood vessels,” J. Cell Sci., Vol.130, No.9, pp. 1583-1595, 2017. https://doi.org/10.1242/jcs.197848
- [37] T. W. Secomb, J. P. Alberding, R. Hsu, M. W. Dewhirst, and A. R. Pries, “Angiogenesis: An adaptive dynamic biological patterning problem,” PLOS Comput. Biol., Vol.9, No.3, Article No.e1002983, 2013. https://doi.org/10.1371/journal.pcbi.1002983
- [38] V. A. Korshunov, S. M. Schwartz, and B. C. Berk, “Vascular remodeling: Hemodynamic and biochemical mechanisms underlying Glagov’s phenomenon,” Arterioscler. Thromb. Vasc. Biol., Vol.27, No.8, pp. 1722-1728, 2007. https://doi.org/10.1161/ATVBAHA.106.129254
- [39] L. D. Browne et al., “The role of shear stress in arteriovenous fistula maturation and failure: A systematic review,” PLoS ONE, Vol.10, No.12, Article No.e0145795, 2015. https://doi.org/10.1371/journal.pone.0145795
- [40] J. D. Humphrey and M. A. Schwartz, “Vascular mechanobiology: Homeostasis, adaptation, and disease,” Annu. Rev. Biomed. Eng., Vol.23, pp. 1-27, 2021. https://doi.org/10.1146/annurev-bioeng-092419-060810
- [41] Q. Chen et al., “Haemodynamics-driven developmental pruning of brain vasculature in zebrafish,” PLOS Biol., Vol.10, No.8, Article No.e1001374, 2012. https://doi.org/10.1371/journal.pbio.1001374
- [42] M. O. Bernabeu et al., “Computer simulations reveal complex distribution of haemodynamic forces in a mouse retina model of angiogenesis,” J. R. Soc. Interface, Vol.11, No.99, Article No.20140543, 2014. https://doi.org/10.1098/rsif.2014.0543
- [43] C. A. Franco et al., “Dynamic endothelial cell rearrangements drive developmental vessel regression,” PLOS Biol., Vol.13, No.4, Article No.e1002125, 2015. https://doi.org/10.1371/journal.pbio.1002125
- [44] H. Sano, M. Watanabe, T. Yamashita, K. Tanishita, and R. Sudo, “Control of vessel diameters mediated by flow-induced outward vascular remodeling in vitro,” Biofabrication, Vol.12, No.4, Article No.045008, 2020. https://doi.org/10.1088/1758-5090/ab9316
- [45] E. Sho et al., “Arterial enlargement in response to high flow requires early expression of matrix metalloproteinases to degrade extracellular matrix.,” Exp. Mol. Pathol., Vol.73, No.2, pp. 142-153, 2002. https://doi.org/10.1006/exmp.2002.2457
- [46] M. A. Patterson et al., “Shear force regulates matrix metalloproteinase activity in human saphenous vein organ culture,” J. Surg. Res., Vol.95, No.1, pp. 67-72, 2001. https://doi.org/10.1006/jsre.2000.6045
This article is published under a Creative Commons Attribution-NoDerivatives 4.0 Internationa License.