Review:
Motor Cortex Plasticity During Functional Recovery Following Brain Damage
Noriyuki Higo
Human Informatics and Interaction Research Institute, National Institute of Advanced Industrial Science and Technology (AIST)
1-1-1 Umezono, Tsukuba, Ibaraki 305-8568, Japan
Although brain damage causes functional impairment, it is often followed by partial or total recovery of function. Recovery is believed to occur primarily because of brain plasticity. Both human and animal studies have significantly contributed to uncovering the neuronal basis of plasticity. Recent advances in brain imaging technology have enabled the investigation of plastic changes in living human brains. In addition, animal experiments have revealed detailed changes at the neural and genetic levels. In this review, plasticity in motor-related areas of the cerebral cortex, which is one of the most well-studied areas of the neocortex in terms of plasticity, is reviewed. In addition, the potential of technological interventions to enhance plasticity and promote functional recovery following brain damage is discussed. Novel neurorehabilitation technologies are expected to be established based on the emerging research on plasticity from the last several decades.
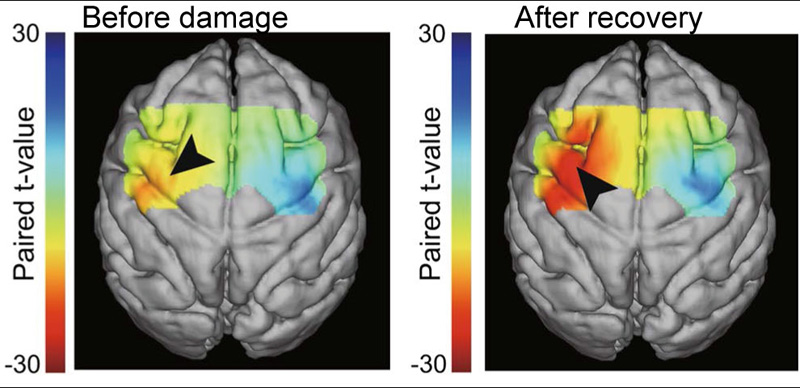
Activation changes during motor recovery from stroke
- [1] E. J. Markus and T. L. Petit, “Synaptic structural plasticity: Role of synaptic shape,” Synapse, Vol.3, No.1, pp. 1-11, 1989.
- [2] R. Lamprecht and J. LeDoux, “Structural plasticity and memory,” Nature Reviews Neuroscience, Vol.5, No.1, pp. 45-54, 2004.
- [3] N. Gogolla, I. Galimberti et al., “Structural plasticity of axon terminals in the adult,” Current Opinion in Neurobiology, Vol.17, No.5, pp. 516-524, 2007.
- [4] A. Holtmaat and K. Svoboda, “Experience-dependent structural synaptic plasticity in the mammalian brain,” Nature Reviews Neuroscience, Vol.10, No.9, pp. 647-658, 2009.
- [5] M. Fu and Y. Zuo, “Experience-dependent structural plasticity in the cortex,” Trends in Neurosciences, Vol.34, No.4, pp. 177-187, 2011.
- [6] P. Caroni, F. Donato et al., “Structural plasticity upon learning: Regulation and functions,” Nature Reviews Neuroscience, Vol.13, No.7, pp. 478-490, 2012.
- [7] A. J. Bower, “Plasticity in the adult and neonatal central nervous system,” British J. of Neurosurgery, Vol.4, No.4, pp. 253-264, 1990.
- [8] P. Calabresi, D. Centonze et al., “Synaptic plasticity in the ischaemic brain,” The Lancet Neurology, Vol.2, No.10, pp. 622-629, 2003.
- [9] F. Boller, “Rational basis of rehabilitation following cerebral lesions: A review of the concept of cerebral plasticity,” Functional Neurology, Vol.19, No.2, pp. 65-72, 2004.
- [10] D. Wang and T. Sun, “Neural plasticity and functional recovery of human central nervous system with special reference to spinal cord injury,” Spinal Cord, Vol.49, No.4, pp. 486-492, 2011.
- [11] G. Balbinot and C. P. Schuch, “Compensatory Relearning Following Stroke: Cellular and Plasticity Mechanisms in Rodents,” Frontiers in Neuroscience, Vol.12, Article No.1023, 2019.
- [12] C. Kelly, J. J. Foxe et al., “Patterns of normal human brain plasticity after practice and their implications for neurorehabilitation,” Archives of Physical Medicine and Rehabilitation, Vol.87, No.12, Supplement, pp. 20-29, 2006.
- [13] J. Tanji, “Sequential organization of multiple movements: Involvement of cortical motor areas,” Annual Review of Neuroscience, Vol.24, pp. 631-651, 2001.
- [14] R. P. Dum and P. L. Strick, “Motor areas in the frontal lobe of the primate,” Physiology & Behavior, Vol.77, Nos.4-5, pp. 677-682, 2002.
- [15] G. Rizzolatti and G. Luppino, “The cortical motor system,” Neuron, Vol.31, No.6, pp. 889-901, 2001.
- [16] N. Higo, N. Kunori et al., “Neural Activity During Voluntary Movements in Each Body Representation of the Intracortical Microstimulation-Derived Map in the Macaque Motor Cortex,” PloS One, Vol.11, No.8, Article No.e0160720, 2016.
- [17] Y. Murata, N. Higo et al., “Temporal plasticity involved in recovery from manual dexterity deficit after motor cortex lesion in macaque monkeys,” J. Neurosci., Vol.35, No.1, pp. 84-95, 2015.
- [18] J. M. Macpherson, C. Marangoz et al., “Microstimulation of the supplementary motor area (SMA) in the awake monkey,” Exp. Brain. Res., Vol.45, No.3, pp. 410-416, 1982.
- [19] R. J. Nudo, G. W. Milliken et al., “Use-dependent alterations of movement representations in primary motor cortex of adult squirrel monkeys,” J. Neurosci., Vol.16, No.2, pp. 785-807, 1996.
- [20] E. J. Plautz, G. W. Milliken et al., “Effects of repetitive motor training on movement representations in adult squirrel monkeys: Role of use versus learning,” Neurobiol. Learn. Mem., Vol.74, No.1, pp. 27-55, 2000.
- [21] A. Kami, G. Meyer et al., “Functional MRI evidence for adult motor cortex plasticity during motor skill learning,” Nature, Vol.377, No.6545, pp. 155-158, 1995.
- [22] R. A. Hodgson, Z. Ji et al., “Training-induced and electrically induced potentiation in the neocortex,” Neurobiol. Learn. Mem., Vol.83, No.1, pp. 22-32, 2005.
- [23] M.-H. Monfils, P. M. VandenBerg et al., “Long-term potentiation induces expanded movement representations and dendritic hypertrophy in layer V of rat sensorimotor neocortex,” Cereb. Cortex, Vol.14, No.5, pp. 586-593, 2004.
- [24] M.-S. Rioult-Pedotti, J. P. Donoghue et al., “Plasticity of the synaptic modification range,” J. Neurophysiol., Vol.98, No.6, pp. 3688-3695, 2007.
- [25] L. Avanzino, N. Gueugneau et al., “Motor cortical plasticity induced by motor learning through mental practice,” Front. Behav. Neurosci., Vol.9, Article No.105, 2015.
- [26] K. Kornysheva and J. Diedrichsen, “Human premotor areas parse sequences into their spatial and temporal features,” Elife, Vol.3, Article No.e03043, 2014.
- [27] X. Lu and J. Ashe, “Anticipatory activity in primary motor cortex codes memorized movement sequences,” Neuron, Vol.45, No.6, pp. 967-973, 2005.
- [28] N. Higo, “Effects of rehabilitative training on recovery of hand motor function: A review of animal studies,” Neuroscience Research, Vol.78, pp. 9-15, 2014.
- [29] Y. Murata, N. Higo et al., “Effects of motor training on the recovery of manual dexterity after primary motor cortex lesion in macaque monkeys,” J. Neurophysiol., Vol.99, No.2, pp. 773-786, 2008.
- [30] N. Kaneko and K. Sawamoto, “Adult neurogenesis and its alteration under pathological conditions,” Neuroscience Research, Vol.63, No.3, pp. 155-164, 2009.
- [31] T. Yamashita, M. Ninomiya et al., “Subventricular zone-derived neuroblasts migrate and differentiate into mature neurons in the post-stroke adult striatum,” J. Neurosci., Vol.26, No.24, pp. 6627-6636, 2006.
- [32] M. Komitova, B. Mattsson et al., “Enriched environment increases neural stem/progenitor cell proliferation and neurogenesis in the subventricular zone of stroke-lesioned adult rats,” Stroke, Vol.36, No.6, pp. 1278-1282, 2005.
- [33] D. R. Kornack and P. Rakic, “Cell proliferation without neurogenesis in adult primate neocortex,” Science, Vol.294, No.5549, pp. 2127-2130, 2001.
- [34] J. W. Fawcett and R. A. Asher, “The glial scar and central nervous system repair,” Brain Research Bulletin, Vol.49, No.6, pp. 377-391, 1999.
- [35] Y.-M. Yuan and C. He, “The glial scar in spinal cord injury and repair,” Neuroscience Bulletin, Vol.29, No.4, pp. 421-435, 2013.
- [36] C. Raposo and M. Schwartz, “Glial scar and immune cell involvement in tissue remodeling and repair following acute CNS injuries,” Glia, Vol.62, No.11, pp. 1895-1904, 2014.
- [37] Y. Liu and E. M. Rouiller, “Mechanisms of recovery of dexterity following unilateral lesion of the sensorimotor cortex in adult monkeys,” Exp. Brain. Res., Vol.128, Nos.1-2, pp. 149-159, 1999.
- [38] A. M. Travis and C. N. Woolsey, “Motor performance of monkeys after bilateral partial and total cerebral decortications,” Am. J. Phys. Med., Vol.35, No.5, pp. 273-310, 1956.
- [39] R. Ogden and S. I. Franz, “On cerebral motor control: The recovery from experimentally produced hemiplegia,” Psychobiology, Vol.1, No.1, pp. 33-49, 1917.
- [40] R. E. Passingham, V. H. Perry et al., “The long-term effects of removal of sensorimotor cortex in infant and adult rhesus monkeys,” Brain, Vol.106, No.3, pp. 675-705, 1983.
- [41] J. A. Vilensky and S. Gilman, “Lesion of the precentral gyrus in nonhuman primates: A pre-medline bibliography,” Int. J. of Primatology, Vol.23, No.6, pp. 1319-1333, 2002.
- [42] R. J. Nudo, B. M. Wise et al., “Neural substrates for the effects of rehabilitative training on motor recovery after ischemic infarct,” Science, Vol.272, No.5269, pp. 1791-1794, 1996.
- [43] B. Alstermark, J. Ogawa et al., “Lack of monosynaptic corticomotoneuronal EPSPs in rats: Disynaptic EPSPs mediated via reticulospinal neurons and polysynaptic EPSPs via segmental interneurons,” J. Neurophysiol., Vol.91, No.4, pp. 1832-1839, 2004.
- [44] G. Courtine, M. B. Bunge et al., “Can experiments in nonhuman primates expedite the translation of treatments for spinal cord injury in humans?,” Nature Medicine, Vol.13, No.5, pp. 561-566, 2007.
- [45] T. Isa, Y. Ohki et al., “Direct and indirect cortico-motoneuronal pathways and control of hand/arm movements,” Physiology, Vol.22, No.2, pp. 145-152, 2007.
- [46] H. G. J. M. Kuypers, “A new look at the organization of the motor system,” Progress in Brain Research, Vol.57, pp. 381-403, 1982.
- [47] R. N. Lemon, “Descending pathways in motor control,” Annual Review of Neuroscience, Vol.31, pp. 195-218, 2008.
- [48] C. Rosso, O. Colliot et al., “Tissue at risk in the deep middle cerebral artery territory is critical to stroke outcome,” Neuroradiology, Vol.53, No.10, Article No.763, 2011.
- [49] S. K. Schiemanck, G. Kwakkel et al., “Impact of internal capsule lesions on outcome of motor hand function at one year post-stroke,” J. of Rehabilitation Medicine, Vol.40, No.2, pp. 96-101, 2008.
- [50] R. Wenzelburger, F. Kopper et al., “Hand coordination following capsular stroke,” Brain, Vol.128, No.1, pp. 64-74, 2005.
- [51] C. Cirillo, A. Le Friec et al., “Focal Malonate Injection into the Internal Capsule of Rats as a Model of Lacunar Stroke,” Front. Neurol., Vol.9, Article No.1072, 2018.
- [52] C.-W. Han, K.-H. Lee et al., “An Experimental Infarct Targeting the Internal Capsule: Histopathological and Ultrastructural Changes,” J. Pathol. Transl. Med., Vol.51, No.3, pp. 292-305, 2017.
- [53] S. Puentes, T. Kaido et al., “Internal capsule stroke in the common marmoset,” Neuroscience, Vol.284, pp. 400-411, 2015.
- [54] Y. Murata and N. Higo, “Development and characterization of a macaque model of focal internal capsular infarcts,” PloS One, Vol.11, No.5, Article No.e0154752, 2016.
- [55] J. Kato, T. Yamada et al., “Functional near-infrared-spectroscopy-based measurement of changes in cortical activity in macaques during post-infarct recovery of manual dexterity,” Sci. Rep., Vol.10, No.1, Article No.6458, 2020.
- [56] U. Horn, S. Roschka et al., “Increased ventral premotor cortex recruitment after arm training in an fMRI study with subacute stroke patients,” Behavioural Brain Research, Vol.308, pp. 152-159, 2016.
- [57] I. Loubinoux, S. Dechaumont-Palacin et al., “Prognostic value of fMRI in recovery of hand function in subcortical stroke patients,” Cereb. Cortex, Vol.17, No.12, pp. 2980-2987, 2007.
- [58] S. Bajaj, S. N. Housley et al., “Dominance of the Unaffected Hemisphere Motor Network and its Role in the Behavior of Chronic Stroke Survivors,” Frontiers in Human Neuroscience, Vol.10, Article No.650, 2016.
- [59] S. Bestmann, O. Swayne et al., “The role of contralesional dorsal premotor cortex after stroke as studied with concurrent TMS-fMRI,” J. Neurosci., Vol.30, No.36, pp. 11926-11937, 2010.
- [60] L. V. Bradnam, C. M. Stinear et al., “Contralesional hemisphere control of the proximal paretic upper limb following stroke,” Cereb. Cortex, Vol.22, No.11, pp. 2662-2671, 2012.
- [61] K. C. Dodd, V. A. Nair et al., “Role of the Contralesional vs. Ipsilesional Hemisphere in Stroke Recovery,” Frontiers in Human Neuroscience, Vol.11, Article No.469, 2017.
- [62] H. Johansen-Berg, M. F. S. Rushworth et al., “The role of ipsilateral premotor cortex in hand movement after stroke,” Proc. of the National Academy of Sciences of the United States of America, Vol.99, No.22, pp. 14518-14523, 2002.
- [63] S. S. Kantak, J. W. Stinear et al., “Rewiring the brain: Potential role of the premotor cortex in motor control, learning, and recovery of function following brain injury,” Neurorehabilitation and Neural Repair, Vol.26, No.3, pp. 282-292, 2012.
- [64] A. K. Rehme, G. R. Fink et al., “The role of the contralesional motor cortex for motor recovery in the early days after stroke assessed with longitudinal fMRI,” Cereb. Cortex, Vol.21, No.4, pp. 756-768, 2011.
- [65] J. D. Schaechter and K. L. Perdue, “Enhanced cortical activation in the contralesional hemisphere of chronic stroke patients in response to motor skill challenge,” Cereb. Cortex, Vol.18, No.3, pp. 638-647, 2008.
- [66] B. Touvykine, B. K. Mansoori et al., “The Effect of Lesion Size on the Organization of the Ipsilesional and Contralesional Motor Cortex,” Neurorehabilitation and Neural Repair, Vol.30, No.3, pp. 280-292, 2016.
- [67] S. Sasaki, T. Isa et al., “Dexterous finger movements in primate without monosynaptic corticomotoneuronal excitation,” J. Neurophysiol., Vol.92, No.5, pp. 3142-3147, 2004.
- [68] Y. Nishimura, H. Onoe et al., “Time-dependent central compensatory mechanisms of finger dexterity after spinal cord injury,” Science, Vol.318, No.5853, pp. 1150-1155, 2007.
- [69] S. T. Carmichael, L. Wei et al., “New patterns of intracortical projections after focal cortical stroke,” Neurobiology of Disease, Vol.8, No.5, pp. 910-922, 2001.
- [70] B. B. Johansson and A.-L. Ohlsson, “Environment, social interaction, and physical activity as determinants of functional outcome after cerebral infarction in the rat,” Experimental Neurology, Vol.139, No.2, pp. 322-327, 1996.
- [71] A.-L. Ohlsson and B. B. Johansson, “Environment influences functional outcome of cerebral infarction in rats,” Stroke, Vol.26, No.4, pp. 644-649, 1995.
- [72] R. Lindenberg, L. L. Zhu et al., “Predicting functional motor potential in chronic stroke patients using diffusion tensor imaging,” Hum. Brain. Mapp., Vol.33, No.5, pp. 1040-1051, 2012.
- [73] S.-H. Jang, “A review of diffusion tensor imaging studies on motor recovery mechanisms in stroke patients,” NeuroRehabilitation, Vol.28, No.4, pp. 345-352, 2011.
- [74] P. R. W. Arachchige, S. Karunarathna et al., “Changes in brain morphometry after motor rehabilitation in chronic stroke,” Somatosens. Mot. Res., Vol.38, No.4, pp. 277-286, 2021.
- [75] K. Chen, Y. Zheng et al., “Exercise training improves motor skill learning via selective activation of mTOR,” Sci. Adv., Vol.5, No.7, Article No.eaaw1888, 2019.
- [76] T. Xu, X. Yu et al., “Rapid formation and selective stabilization of synapses for enduring motor memories,” Nature, Vol.462, No.7275, pp. 915-919, 2009.
- [77] V. C. K. Cheung, C. Deboer et al., “Gene expression changes in the motor cortex mediating motor skill learning,” PloS One, Vol.8, No.4, Article No.e61496, 2013.
- [78] R. H. Roth, R. H. Cudmore et al., “Cortical Synaptic AMPA Receptor Plasticity During Motor Learning,” Neuron, Vol.105, No.5, pp. 895-908, 2020.
- [79] M. T. Hasan, S. Hernández-González et al., “Role of motor cortex NMDA receptors in learning-dependent synaptic plasticity of behaving mice,” Nat. Commun., Vol.4, Article No.2258, 2013.
- [80] S. Li, J. J. Overman et al., “An age-related sprouting transcriptome provides molecular control of axonal sprouting after stroke,” Nature Neuroscience, Vol.13, No.12, pp. 1496-1504, 2010.
- [81] J. Kaiser, M. Maibach et al., “The Spinal Transcriptome After Cortical Stroke: In Search of Molecular Factors Regulating Spontaneous Recovery in the Spinal Cord,” J. Neurosci., Vol.39, No.24, pp. 4714-4726, 2019.
- [82] M. Ito, M. Aswendt et al., “RNA-Sequencing Analysis Revealed a Distinct Motor Cortex Transcriptome in Spontaneously Recovered Mice After Stroke,” Stroke, Vol.49, No.9, pp. 2191-2199, 2018.
- [83] J.-B. Kim, C.-S. Piao et al., “Delayed genomic responses to transient middle cerebral artery occlusion in the rat,” J. of Neurochemistry, Vol.89, No.5, pp. 1271-1282, 2004.
- [84] A. Rönnbäck, P. Dahlqvist et al., “Gene expression profiling of the rat hippocampus one month after focal cerebral ischemia followed by enriched environment,” Neuroscience Letters, Vol.385, No.2, pp. 173-178, 2005.
- [85] K. Mizutani, S. Sonoda et al., “Alteration of protein expression profile following voluntary exercise in the perilesional cortex of rats with focal cerebral infarction,” Brain Research, Vol.1416, pp. 61-68, 2011.
- [86] J.-H. Yi, S.-W. Park et al., “Role of transcription factors in mediating post-ischemic cerebral inflammation and brain damage,” Neurochemistry Int., Vol.50, Nos.7-8, pp. 1014-1027, 2007.
- [87] M. Ahmad, N. J. Dar et al., “Inflammation in ischemic stroke: Mechanisms, consequences and possible drug targets,” CNS & Neurological Disorders – Drug Targets, Vol.13, No.8, pp. 1378-1396, 2014.
- [88] Z. Zheng, J. E. Lee et al., “Stroke: Molecular mechanisms and potential targets for treatment,” Current Molecular Medicine, Vol.3, No.4, pp. 361-372, 2003.
- [89] G. Ford, Z. Xu et al., “Expression analysis systematic explorer (EASE) analysis reveals differential gene expression in permanent and transient focal stroke rat models,” Brain Research, Vol.1071, No.1, pp. 226-236, 2006.
- [90] J. J. Velier, J. A. Ellison et al., “Caspase-8 and caspase-3 are expressed by different populations of cortical neurons undergoing delayed cell death after focal stroke in the rat,” J. Neurosci., Vol.19, No.14, pp. 5932-5941, 1999.
- [91] J.-C. Delpech, C. Madore et al., “Microglia in neuronal plasticity: Influence of stress,” Neuropharmacology, Vol.96, Part A, pp. 19-28, 2015.
- [92] G. P. Morris, I. A. Clark et al., “Microglia: A new frontier for synaptic plasticity, learning and memory, and neurodegenerative disease research,” Neurobiol. Learn. Mem., Vol.105, pp. 40-53, 2013.
- [93] U. B. Eyo and M. E. Dailey, “Microglia: Key elements in neural development, plasticity, and pathology,” J. of Neuroimmune Pharmacology, Vol.8, No.3, pp. 494-509, 2013.
- [94] K.-H. Kim, S.-M. Son et al., “Contributions of microglia to structural synaptic plasticity,” J. of Experimental Neuroscience, Vol.7, pp. 85-91, 2013.
- [95] D. P. Schafer and B. Stevens, “Microglia Function in Central Nervous System Development and Plasticity,” Cold Spring Harbor Perspectives in Biology, Vol.7, No.10, Article No.a020545, 2015.
- [96] I. Sandvig, I. L. Augestad et al., “Neuroplasticity in stroke recovery. The role of microglia in engaging and modifying synapses and networks,” European J. of Neuroscience, Vol.47, No.12, pp. 1414-1428, 2018.
- [97] Y. Zhao and D. A. Rempe, “Targeting astrocytes for stroke therapy,” Neurotherapeutics, Vol.7, No.4, pp. 439-451, 2010.
- [98] S.-Y. Kim, J. E. Hsu et al., “Coordinated Plasticity of Synapses and Astrocytes Underlies Practice-Driven Functional Vicariation in Peri-Infarct Motor Cortex,” J. Neurosci., Vol.38, No.1, pp. 93-107, 2018.
- [99] Y. Chen and R. A. Swanson, “Astrocytes and brain injury,” J. of Cerebral Blood Flow and Metabolism, Vol.23, No.2, pp. 137-149, 2003.
- [100] A. J. Barker and E. M. Ullian, “Astrocytes and synaptic plasticity,” The Neuroscientist, Vol.16, No.1, pp. 40-50, 2010.
- [101] M. De Pitta, N. Brunel et al., “Astrocytes: Orchestrating synaptic plasticity?,” Neuroscience, Vol.323, pp. 43-61, 2016.
- [102] S. T. Carmichael, I. Archibeque et al., “Growth-associated gene expression after stroke: Evidence for a growth-promoting region in peri-infarct cortex,” Experimental Neurology, Vol.193, No.2, pp. 291-311, 2005.
- [103] N. Higo, T. Oishi et al., “Gene expression of growth-associated proteins, GAP-43 and SCG10, in the hippocampal formation of the macaque monkey: Nonradioactive in situ hybridization study,” Hippocampus, Vol.8, No.5, pp. 533-547, 1998.
- [104] L. I. Benowitz and A. Routtenberg, “GAP-43: An intrinsic determinant of neuronal development and plasticity,” Trends in Neurosciences, Vol.20, No.2, pp. 84-91, 1997.
- [105] M. E. Schwab, “Structural plasticity of the adult CNS. Negative control by neurite growth inhibitory signals,” Int. J. Dev. Neurosci., Vol.14, No.4, pp. 379-385, 1996.
- [106] N. Dancause, S. Barbay et al., “Extensive cortical rewiring after brain injury,” J. Neurosci., Vol.25, No.44, pp. 10167-10179, 2005.
- [107] T. Yamamoto, T. Hayashi et al., “Premotor Cortical-Cerebellar Reorganization in a Macaque Model of Primary Motor Cortical Lesion and Recovery,” J. Neurosci., Vol.39, No.43, pp. 8484-8496, 2019.
- [108] Y. Murata, N. Higo et al., “Increased expression of the growth-associated protein-43 gene after primary motor cortex lesion in macaque monkeys,” Neuroscience Research, Vol.98, pp. 64-69, 2015.
- [109] H. Nakagawa, T. Ninomiya et al., “Reorganization of corticospinal tract fibers after spinal cord injury in adult macaques,” Sci. Rep., Vol.5, Article No.11986, 2015.
- [110] E. S. Rosenzweig, G. Courtine et al., “Extensive spontaneous plasticity of corticospinal projections after primate spinal cord injury,” Nature Neuroscience, Vol.13, No.12, pp. 1505-1510, 2010.
- [111] N. Higo, Y. Nishimura et al., “Increased expression of the growth-associated protein 43 gene in the sensorimotor cortex of the macaque monkey after lesioning the lateral corticospinal tract,” The J. of Comparative Neurology, Vol.516, No.6, pp. 493-506, 2009.
- [112] N. Higo, A. Sato et al., “Comprehensive analysis of area-specific and time-dependent changes in gene expression in the motor cortex of macaque monkeys during recovery from spinal cord injury,” The J. of Comparative Neurology, Vol.526, No.7, pp. 1110-1130, 2018.
- [113] N. Higo, A. Sato et al., “SPP1 is expressed in corticospinal neurons of the macaque sensorimotor cortex,” The J. of Comparative Neurology, Vol.518, No.13, pp. 2633-2644, 2010.
- [114] T. Yamamoto, T. Oishi et al., “Differential expression of secreted phosphoprotein 1 in the motor cortex among primate species and during postnatal development and functional recovery,” PloS One, Vol.8, No.5, Article No.e65701, 2013.
- [115] S. B. Frost, S. Barba et al., “Reorganization of remote cortical regions after ischemic brain injury: A potential substrate for stroke recovery,” J. Neurophysiol., Vol.89, No.6, pp. 3205-3214, 2003.
- [116] M. P. Galea and I. Darian-Smith, “Multiple corticospinal neuron populations in the macaque monkey are specified by their unique cortical origins, spinal terminations, and connections,” Cereb. Cortex, Vol.4, No.2, pp. 166-194, 1994.
- [117] M. A. Maldonado, R. P. Allred et al., “Motor skill training, but not voluntary exercise, improves skilled reaching after unilateral ischemic lesions of the sensorimotor cortex in rats,” Neurorehabilitation and Neural Repair, Vol.22, No.3, pp. 250-261, 2008.
- [118] J. Biernaskie and D. Corbett, “Enriched rehabilitative training promotes improved forelimb motor function and enhanced dendritic growth after focal ischemic injury,” J. Neurosci., Vol.21, No.14, pp. 5272-5280, 2001.
- [119] J. Biernaskie, G. Chernenko et al., “Efficacy of rehabilitative experience declines with time after focal ischemic brain injury,” J. Neurosci., Vol.24, No.5, pp. 1245-1254, 2004.
- [120] Y. Sugiyama, N. Higo et al., “Effects of early versus late rehabilitative training on manual dexterity after corticospinal tract lesion in macaque monkeys,” J. Neurophysiol., Vol.109, No.12, pp. 2853-2865, 2013.
- [121] D. W. Choi, J.-Y. Koh et al., “Pharmacology of glutamate neurotoxicity in cortical cell culture: Attenuation by NMDA antagonists,” J. Neurosci., Vol.8, No.1, pp. 185-196, 1988.
- [122] D. W. Choi, M. Maulucci-Gedde et al., “Glutamate neurotoxicity in cortical cell culture,” J. Neurosci., Vol.7, No.2, pp. 357-368, 1987.
- [123] J. L. Humm, D. A. Kozlowski et al., “Use-dependent exaggeration of brain injury: Is glutamate involved?,” Experimental Neurology, Vol.157, No.2, pp. 349-358, 1999.
- [124] R. L. Michaels and S. M. Rothman, “Glutamate neurotoxicity in vitro: Antagonist pharmacology and intracellular calcium concentrations,” J. Neurosci., Vol.10, No.1, pp. 283-292, 1990.
- [125] W.-T. Chien, Y.-Y. Chong et al., “Robot-assisted therapy for upper-limb rehabilitation in subacute stroke patients: A systematic review and meta-analysis,” Brain. Behav., Vol.10, No.8, Article No.e01742, 2020.
- [126] E. López-Larraz, A. Sarasola-Sanz et al., “Brain-machine interfaces for rehabilitation in stroke: A review,” NeuroRehabilitation, Vol.43, No.1, pp. 77-97, 2018.
- [127] A. Biasiucci, R. Leeb et al., “Brain-actuated functional electrical stimulation elicits lasting arm motor recovery after stroke,” Nat. Commun., Vol.9, No.1, Article No.2421, 2018.
- [128] S. Liebigt, N. Schlegel et al., “Effects of rehabilitative training and anti-inflammatory treatment on functional recovery and cellular reorganization following stroke,” Experimental Neurology, Vol.233, No.2, pp. 776-782, 2012.
- [129] T. Wang, J.-Q. Xiong et al., “The role of Nogo-A in neuroregeneration: A review,” Brain Research Bulletin, Vol.87, No.6, pp. 499-503, 2012.
- [130] R. J. McKeon, R. C. Schreiber et al., “Reduction of neurite outgrowth in a model of glial scarring following CNS injury is correlated with the expression of inhibitory molecules on reactive astrocytes,” J. Neurosci., Vol.11, No.11, pp. 3398-3411, 1991.
- [131] L. C. Smith-Thomas, J. Fok-Seang et al., “An inhibitor of neurite outgrowth produced by astrocytes,” J. Cell. Sci., Vol.107, No.6, pp. 1687-1695, 1994.
- [132] S. J. A. Davies, D. R. Goucher et al., “Robust regeneration of adult sensory axons in degenerating white matter of the adult rat spinal cord,” J. Neurosci., Vol.19, No.14, pp. 5810-5822, 1999.
- [133] T. GrandPré, S. Li et al., “Nogo-66 receptor antagonist peptide promotes axonal regeneration,” Nature, Vol.417, No.6888, pp. 547-551, 2002.
- [134] E. J. Bradbury, L. D. F. Moon et al., “Chondroitinase ABC promotes functional recovery after spinal cord injury,” Nature, Vol.416, No.6881, pp. 636-640, 2002.
- [135] H. Abe, S. Jitsuki et al., “CRMP2-binding compound, edonerpic maleate, accelerates motor function recovery from brain damage,” Science, Vol.360, No.6384, pp. 50-57, 2018.
- [136] J. M. Conner, A. A. Chiba et al., “The basal forebrain cholinergic system is essential for cortical plasticity and functional recovery following brain injury,” Neuron, Vol.46, No.2, pp. 173-179, 2005.
- [137] J. M. Conner, A. Culberson et al., “Lesions of the basal forebrain cholinergic system impair task acquisition and abolish cortical plasticity associated with motor skill learning,” Neuron, Vol.38, No.5, pp. 819-829, 2003.
- [138] D. F. Goncalves, M. S. Guzman et al., “Striatal Acetylcholine Helps to Preserve Functional Outcomes in a Mouse Model of Stroke,” ASN Neuro, Vol.12, 2020.
- [139] F. Hummel and L. G. Cohen, “Improvement of motor function with noninvasive cortical stimulation in a patient with chronic stroke,” Neurorehabilitation and Neural Repair, Vol.19, No.1, pp. 14-19, 2005.
- [140] P. S. Boggio, A. Nunes et al., “Repeated sessions of noninvasive brain DC stimulation is associated with motor function improvement in stroke patients,” Restorative Neurology and Neuroscience, Vol.25, No.2, pp. 123-129, 2007.
- [141] F. Fregni, P. S. Boggio et al., “Transcranial direct current stimulation of the unaffected hemisphere in stroke patients,” NeuroReport, Vol.16, No.14, pp. 1551-1555, 2005.
- [142] S. Hesse, A. Waldner et al., “Combined transcranial direct current stimulation and robot-assisted arm training in subacute stroke patients: An exploratory, randomized multicenter trial,” Neurorehabilitation and Neural Repair, Vol.25, No.9, pp. 838-846, 2011.
- [143] M. A. Nitsche and W. Paulus, “Excitability changes induced in the human motor cortex by weak transcranial direct current stimulation,” The J. of Physiology, Vol.527, No.3, pp. 633-639, 2000.
- [144] T. Shimizu, A. Hosaki et al., “Motor cortical disinhibition in the unaffected hemisphere after unilateral cortical stroke,” Brain, Vol.125, No.8, pp. 1896-1907, 2002.
- [145] P. Cicinelli, P. Pasqualetti et al., “Interhemispheric asymmetries of motor cortex excitability in the postacute stroke stage: A paired-pulse transcranial magnetic stimulation study,” Stroke, Vol.34, No.11, pp. 2653-2658, 2003.
- [146] R. Polanía, W. Paulus et al., “Reorganizing the intrinsic functional architecture of the human primary motor cortex during rest with non-invasive cortical stimulation,” PloS One, Vol.7, No.1, Article No.e30971, 2012.
- [147] E. J. Plautz, S. Barbay et al., “Post-infarct cortical plasticity and behavioral recovery using concurrent cortical stimulation and rehabilitative training: A feasibility study in primates,” Neurological Research, Vol.25, No.8, pp. 801-810, 2003.
- [148] C. J. Stagg, J. G. Best et al., “Polarity-sensitive modulation of cortical neurotransmitters by transcranial stimulation,” J. Neurosci., Vol.29, No.16, pp. 5202-5206, 2009.
- [149] B. Fritsch, J. Reis et al., “Direct current stimulation promotes BDNF-dependent synaptic plasticity: Potential implications for motor learning,” Neuron, Vol.66, No.2, pp. 198-204, 2010.
- [150] N. Higo, T. Oishi et al., “Expression of GAP-43 and SCG10 mRNAs in lateral geniculate nucleus of normal and monocularly deprived macaque monkeys,” J. Neurosci., Vol.20, No.16, pp. 6030-6038, 2000.
- [151] N. Higo, “Non-Human Primate Models to Explore the Adaptive Mechanisms After Stroke,” Front. Syst. Neurosci., Vol.15, Article No.760311, 2021.
- [152] R. Heffner and B. Masterton, “Variation in form of the pyramidal tract and its relationship to digital dexterity,” Brain. Behav. Evol., Vol.12, No.3, pp. 161-200, 1975.
- [153] R. S. Heffner and R. B. Masterton, “The role of the corticospinal tract in the evolution of human digital dexterity,” Brain. Behav. Evol., Vol.23, Nos.3-4, pp. 165-183, 1983.
- [154] F. M. Krienen, M. Goldman et al., “Innovations present in the primate interneuron repertoire,” Nature, Vol.586, No.7828, pp. 262-269, 2020.
- [155] L. Geirsdottir, E. David et al., “Cross-Species Single-Cell Analysis Reveals Divergence of the Primate Microglia Program,” Cell, Vol.179, No.7, pp. 1609-1622.e16, 2019.
- [156] D. C. Van Essen, C. J. Donahue et al., “Cerebral cortical folding, parcellation, and connectivity in humans, nonhuman primates, and mice,” Proc. of the National Academy of Sciences of the United States of America, Vol.116, No.52, pp. 26173-26180, 2019.
- [157] M. A. Marin and S. T. Carmichael, “Mechanisms of demyelination and remyelination in the young and aged brain following white matter stroke,” Neurobiology of Disease, Vol.126, pp. 5-12, 2019.
- [158] J. Kato, Y. Murata et al., “Time- and area-dependent macrophage/microglial responses after focal infarction of the macaque internal capsule,” Neuroscience Research, Vol.170, pp. 350-359, 2021.
- [159] G. Wang, J. Zhang et al., “Microglia/macrophage polarization dynamics in white matter after traumatic brain injury,” J. of Cerebral Blood Flow and Metabolism, Vol.33, No.12, pp. 1864-1874, 2013.
- [160] H.-G. Yeo, J.-J. Hong et al., “Increased CD68/TGFβ Co-Expressing Microglia/Macrophages After Transient Middle Cerebral Artery Occlusion in Rhesus Monkeys,” Exp. Neurobiol., Vol.28, No.4, pp. 458-473, 2019.
- [161] W. P. Yew, N. D. Djukic et al., “Early treatment with minocycline following stroke in rats improves functional recovery and differentially modifies responses of peri-infarct microglia and astrocytes,” J. Neuroinflammation, Vol.16, No.1, Article No.6, 2019.
- [162] K. Nagasaka, K. Yamanaka et al., “Brain activity changes in a macaque model of oxaliplatin-induced neuropathic cold hypersensitivity,” Sci. Rep., Vol.7, No.1, Article No.4305, 2017.
- [163] A. Thiel, B. A. Radlinska et al., “The temporal dynamics of poststroke neuroinflammation: A longitudinal diffusion tensor imaging-guided PET study with 11C-PK11195 in acute subcortical stroke,” J. Nucl. Med., Vol.51, No.9, pp. 1404-1412, 2010.
- [164] E. E. Nelson and J. T. Winslow, “Non-human primates: Model animals for developmental psychopathology,” Neuropsychopharmacology, Vol.34, No.1, pp. 90-105, 2009.
- [165] N. Palomero-Gallagher and K. Zilles, “Differences in cytoarchitecture of Broca’s region between human, ape and macaque brains,” Cortex, Vol.118, pp. 132-153, 2018.
- [166] C. Xu, Q. Li et al., “Human-specific features of spatial gene expression and regulation in eight brain regions,” Genome Research, Vol.28, No.8, pp. 1097-1110, 2018.
- [167] T. E. Bakken, J. A. Miller et al., “A comprehensive transcriptional map of primate brain development,” Nature, Vol.535, No.7612, pp. 367-375, 2016.
- [168] Z. He, D. Han et al., “Comprehensive transcriptome analysis of neocortical layers in humans, chimpanzees and macaques,” Nature Neuroscience, Vol.20, No.6, pp. 886-895, 2017.
- [169] S. D. Iversen and L. Weiskrantz, “Temporal Lobe Lesions and Memory in the Monkey,” Nature, Vol.201, No.4920, pp. 740-742, 1964.
- [170] Y. Miyashita, “Inferior temporal cortex: Where visual perception meets memory,” Annual Review of Neuroscience, Vol.16, pp. 245-263, 1993.
- [171] N. Higo, T. Oishi et al., “Quantitative non-radioactive in situ hybridization study of GAP-43 and SCG10 mRNAs in the cerebral cortex of adult and infant macaque monkeys,” Cereb. Cortex, Vol.9, No.4, pp. 317-331, 1999.
- [172] N. Higo, T. Oishi et al., “Northern blot and in situ hybridization analyses of MARCKS mRNA expression in the cerebral cortex of the macaque monkey,” Cereb. Cortex, Vol.12, No.5, pp. 552-564, 2002.
- [173] N. Higo, T. Oishi et al., “Expression of MARCKS mRNA in lateral geniculate nucleus and visual cortex of normal and monocularly deprived macaque monkeys,” Vis. Neurosci., Vol.19, No.5, pp. 633-643, 2002.
- [174] Y. Murata, N. Higo et al., “Developmental changes in the expression of growth-associated protein-43 mRNA in the monkey thalamus: Northern blot and in situ hybridization studies,” Neuroscience, Vol.136, No.2, pp. 497-507, 2005.
This article is published under a Creative Commons Attribution-NoDerivatives 4.0 Internationa License.